Mechanical and Materials Engineering
Scott Schrage, May 21, 2020
Study yields breakthroughs in understanding failure of high-performance fibers
Pull ’n’ peel.
For many, the phrase probably conjures the signature bundles of red licorice (and the only real way to eat them). To material scientists like the University of Nebraska–Lincoln’s Yuris Dzenis and his colleagues, though, it represents a useful metaphor for the surprisingly similar structure of the high-performance fibers found in body armor and aerospace engineering.
It could also describe a powerful new technique for analyzing and, Dzenis hopes, ultimately combating the failure of those polymer fibers — not a decade too soon.
The 1960s and ’70s unleashed a deluge of fiber-related advances, what Dzenis termed “a true revolution” in improving their chemistry, composition and processing. But that wellspring dried up in 1980s, he said, and has remained relatively barren since.
One probable bottleneck? A limited grasp on how fibers behave when getting stretched to their breaking point, otherwise known as tensile strength.
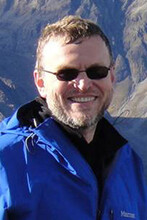
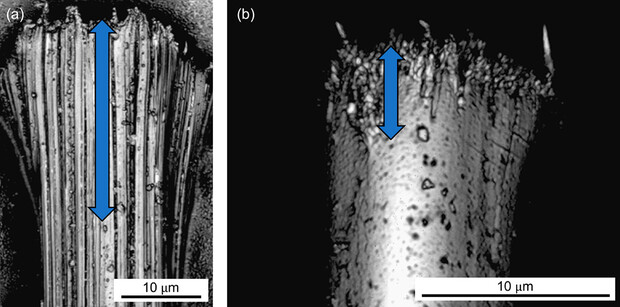
“What we think, and our U.S. Army collaborators also think, is that it may be due to our poor understanding of how these complex fibers respond to loading,” said Dzenis, McBroom Professor of mechanical and materials engineering. “Despite the fact that they’ve been studied for five decades, there’s still no full understanding of fracture mechanisms and deformation.
“As always, when we want to optimize something, we need to understand it first.”
Material scientists already understood that a high-performance fiber generally consists of three hierarchies: nanoscopic tendrils that are thousands of times thinner than human hair; microscopic, tightly packed bundles of those tendrils; and the macroscopic fiber that those bundles make up. Or, in pull ’n’ peel terms: individual strands of licorice, the bundles those strands are pulled from, and the package that contains them.
Though researchers had analyzed how fibers responded on the nano- and macroscale, none had figured out how to measure the interactions among the microscopic bundles — interactions that many suspected were critical to understanding some counterintuitive findings and the process overall.
Dzenis-supervised doctoral graduate Taylor Stockdale and colleagues at the U.S. Army Research Laboratory were up to the task. Stockdale devised a technique to etch miniscule T-shaped notches into the top of the fiber and peel back its surface while it was being stretched, all while avoiding the perturbations that invalidated measurements captured by other techniques — the nanoscopic equivalent of walking a tightrope without disturbing it. With the fiber’s guts revealed, the team was then able to employ more familiar methods, using a nano-indenting instrument to measure the forces separating adjacent bundles and a sophisticated microscope to image those bundles tearing apart.
Having done that, the team set out to compare the behavior of two common high-performance fibers: a Kevlar fiber consisting of rigid polymer chains and another, more flexible polyethylene fiber. Dzenis and his colleagues were especially interested in analyzing fiber fibrillation, the tendency of bundles to tear not at the same point — as in a clean break — but at different points along the length of a fiber, leading to bundle pullout and fiber failure. Because no team had ever managed to quantify the separation among bundles, that process, much like the bundles themselves, had remained hidden beneath the surface.
The team’s experiments revealed that substantially less energy was needed to separate bundles in the flexible-chain polyethylene fiber than in the stiffer Kevlar fiber, helping clarify why fibrillations propagated much farther along the length of the former fibers than the latter.
That resulting data, and the technique that yielded it, should inform future computational models and eventually help optimize manufacturing processes that lead to more-resistant, longer-lasting fibers, the researchers said.American Chemical Society“For the first time, this information allowed us to explain the differences in fibrillation,” Dzenis said of the team’s study, which recently graced the cover of the journal ACS Applied Materials and Interfaces. “We’re explaining the differences through data, which is already a big breakthrough.”
It wasn’t the only one. After comparing the amount of absorbed separation energy at all three scales of the polyethylene fiber — tendril, bundle and entire fiber — the team found that the energy obeyed a so-called power law. In this case, the absorbed separation energy seemed to increase proportionally with the separation surface area taken to the power of about 0.5, meaning that the energy increased at a consistently slowing rate relative to the increase in scale. That, in turn, suggested that tendrils should be easier to separate than bundles, and bundles easier than entire fibers.
And that wasn’t all. In structures, power-law scaling is often accompanied by self-similarity: a phenomenon in which parts of a structure resemble the structure as a whole, as when the arms of a snowflake share structural features with the entire flake. Sure enough, when the team compared images of separation fracture among the microscopic bundles and the macroscopic fiber parts, it spotted similar bridges of material spanning the gaps at both scales — evidence of self-similarity that might also help explain the power-law scaling.
“People in fracture mechanics, in physics, they usually celebrate when they see something like this, because it’s so rich for future modeling and so on,” Dzenis said. “It’s also very fundamental. It may end up being at the core of this complex, multiscale fracture behavior.
“We anticipate people will now be looking for self-similarity in fibers, probably for the first time, because there was no evidence of anything like this before. There was a missing link. Now we have it.”
Questions do remain, Dzenis said, the most intriguing of which relates to the adage of a chain breaking at its weakest link. That adage usually does apply to the failure of structures, he said. Given the team’s findings on absorbed separation energy, the principle suggested that the worst fibrillation should have occurred among the nanoscopic tendrils, not the microscopic bundles.
“That puzzled us,” he said. “That actually delayed the publication for about half a year. We were going back and forth; we had probably 15 drafts of this paper until we settled on this thing. According to the energy, the fibrillation should have been at the nanoscale. But something precludes it in the fiber, and the major fibrillation is at the intermediate scale. The answer to this question is yet to be formulated or finalized, but we have some clues now.”
In the meantime, Dzenis said, the team’s multiple breakthroughs should help material scientists and engineers at least begin unraveling some of the major threads that have confined the field for so long.
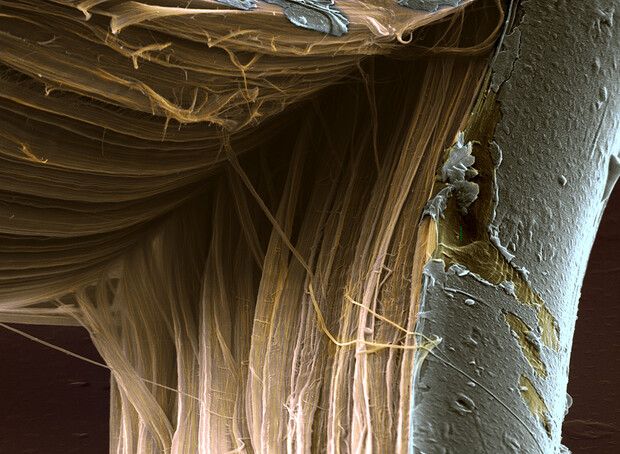
“Fiber manufacturing processes are complex and still not well-understood, but some things can be tweaked: some concentrations, some chemistry, some draw ratios and temperatures and so forth,” he said. “When we change them, we can measure a final fiber property, but with such a complex failure mechanism … the final property is just one data point. It’s not enough to understand how or why a change in processing will affect the failure mechanism. Better understanding of details on different scales will be very, very powerful and beneficial information for researchers. This quantitative information is the key to further developing those mechanisms and creating new super-fibers.
“We’re excited. Not often do you find something qualitatively new, let alone quantitatively unexpected. But this is just the beginning.”
Dzenis, Stockdale and Nebraska postdoctoral researcher Dimitry Papkov authored the study with the U.S. Army Research Lab’s Kenneth Strawhecker, Daniel Cole, Jeffrey Staniszewski, Michael Roenbeck and Steve Lustig. The team received support from the Army Research Lab, the National Science Foundation, the Office of Naval Research, the National Institutes of Health and the Nebraska Center for Energy Science Research.